9.1.1 Upper-Air
Meteorological Variables
Meteorological
variables measured/reported in upper-air monitoring programs include wind
direction, wind speed, pressure, temperature, and humidity. With some
exceptions (e.g., radiosonde measurements of pressure, temperature, and
humidity), the upper-air data for these variables are based on indirect
measurements; i.e., the desired variable is derived from measurements of
other variables which are measured directly. This is a significant
difference from the in situ measurements of these variables; i.e., when
monitored in situ (such as from a meteorological tower) these variables are
measured directly. This difference has significant implications for
calibrations and audits of upper-air measurement systems (see Section 9.6).
Fundamentals
related to upper-air monitoring of wind, pressure, temperature, and humidity
are presented in the following. This is followed by information on
estimating mixing heights and stability for use in dispersion modeling.
Although the latter are often included in discussions of upper-air
meteorological conditions, they are not really upper-air variables; a more accurate classification of mixing height would describe it as a boundary
layer variable which can be derived from upper-air measurements. Stability,
as defined for use in dispersion modeling, is a surface layer variable and
is not necessarily related to or correlated with upper-air measurements.
Wind
Upper-air wind speeds and wind
directions are vector-averaged measurements. None of the measurement systems
described in the following sections provide a means to measure
winds as scaler quantities, as is done with cup and vane sensors mounted on
aninstrumented tower. While tower-based
measurements near the surface are easily obtained, there are very few
instrumented tall towers that can provide vertical profiles of upper-air
winds over the altitudes needed for some air quality applications.
Upper-air
wind data comprise either path averages (radiosondes) or volume averages (remote
sensors) rather than point measurements. For air quality programs, where the
interest is mainly to characterize winds in the atmospheric boundary layer (ABL)
and lower troposphere, radiosonde data are typically averaged over vertical
layers with a depth of approximately 45 to 75 meters (m). Wind data provided
by sodars are typically averaged over layers that are 5 to 100 m deep, while
radar wind profiler data are usually averaged over 60 to 100 meter
intervals. The altitude at which the winds are reported is assumed to be the
mid-point of the layer over which the winds are averaged. Averaging periods
for upper-air wind data also vary depending on the instrument system used.
An individual wind data report from a radiosonde sounding system is
typically averaged over no more than 30 to 120 seconds, representing
averages of 60 to 700 meters. The averaging interval for winds measured by
sodars and radar profilers is usually on the order of 15 to 60 minutes.
Upper-air
wind data are needed to accurately characterize upper-air transport. For example,
observing and resolving the vertical shear of the horizontal wind (both
speed and directional changes with height) can be important for air quality
model applications. Figure 9-1 shows a plot of upper-air winds measured by a
radiosonde sounding system, along with simultaneous profiles of temperature,
dew-point temperature, and potential temperature. The wind data are
represented in the “wind barb” format, in which the direction of the
wind is indicated by the orientation of an arrow's shaft (relative to true
north, which is toward the top of the figure), and the wind speed is
indicated by the number and length of barbs attached to the shaft. Note the
change in wind speed and direction that is evident in the first few hundred
meters of the sounding. In this case, below about 280 meters the winds are
east-southeasterly. Above this level the winds veer (turn clockwise) with
height to become southerly, southwesterly, then westerly. This is a simple
example of a pattern that is common in upper-air measurements; in fact, much
more complex wind shear conditions are often observed. Wind shear conditions
can have important implications with respect to air quality, because of the
different transport and turbulence conditions that can exist at different
altitudes where air pollutants may be present.
Shear
patterns such as those depicted in Figure 9-1 occur in part because of the
frictional drag exerted on the atmosphere
by the earth's surface. The atmospheric boundary layer isgenerally
defined as the layer of the atmosphere within which the dynamic properties
(i.e., winds) and thermodynamic properties (i.e., temperature, pressure,
moisture) are directly influenced by the earth's surface. Factors that
influence the vertical distribution of winds include
- horizontal
gradients in temperature (thermal wind effects),
- the
development of local temperature and pressure gradients in shoreline
settings (land/sea-breeze circulations) and complex terrain environments
(mountain-valley airflows)
- vertical
momentum transport by turbulent eddies,
- diurnal
reductions in frictional stress at night that can lead to the formation
of low-level jets.
Processes
such as these are described in references [68] and [60]; examples of the
effects of such circulations on air quality are described in reference [70].
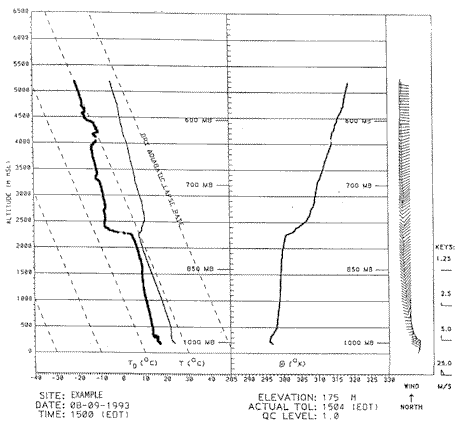
Figure
9-1 Example wind and temperature profiles from a radiosonde sounding system.
Consequently,
upper-air wind data are critical to air quality analysis and modeling
efforts. The data are used for the
assessment of transport characteristics, as direct input to Gaussian
dispersion models, and in the initialization and application of
meteorological models (that are used to prepare time-varying,
three-dimensional meteorological fields for puff and grid-based air quality
models).
Upper-air
wind speeds are almost always reported in units of meters per second (ms -1
) or knots (nautical miles per hour).
Wind direction is reported as the direction from which the wind is blowing
in degrees (clockwise) relative to true north. Altitude is usually reported
in meters or feet and must be defined as corresponding to height above mean
sea level or height above ground level. Radiosonde data are typically
reported as height above mean sea level (msl), whereas wind data collected
by the remote sensing systems are often reported as height above ground
level (agl).
Some
remote sensing systems described in these guidelines provide a measure of
vertical velocity. To date, however,
little use has been made of these data in air quality modeling or data
analysis applications. Additional work is needed (possibly on a case-by-case
basis) to determine the utility of these data for air quality applications.
Pressure
Vertical profiles of atmospheric
pressure are measured during radiosonde ascents.
The remote sensing systems considered in this document do not measure
pressure. Pressure data are critical for radiosonde soundings because they
are used to calculate the altitude of the sonde (strictly speaking, the
geopotential altitude). Differential global position systems (GPS)
rawinsonde systems are being developed that will be able to measure the
altitude of the sonde directly, but pressure data will still be needed to
support many modeling and data analysis efforts. For air quality purposes,
pressure data are used in the application of meteorological models, and as
direct input to air quality models. Pressure is reported in units of
millibars (mb) or hectopascals (hPa).
Temperature
Upper-air temperature measurements
are most commonly obtained using radiosonde sounding systems. Radiosonde
temperature measurements are point measurements. These can be obtained every
few seconds, yielding a vertical resolution of a few meters to about 10 m,
depending on the rate of ascent of the balloon.
Temperature
data can also be obtained using RASS. RASS temperature measurementsare
volume averages, with a vertical resolution comparable to that of the wind
measurements reported by the remote
sensing systems (i.e., 50 to 100 m). RASS measures the virtual temperature (Tv
) of the air rather than the dry-bulb temperature (T). The virtual
temperature of an air parcel is the temperature that dry air would have if
its pressure and density were equal to those of a parcel of moist air, and
thus Tv is always higher than the dry-bulb temperature. Under hot and humid
conditions, the difference between Tv and T is usually on the order of a few
(2 to 3) degrees C; at low humidity, differences between Tv and T are small.
Given representative moisture and pressure profiles, temperature can be
estimated from the virtual temperature measurements.
Temperature
data are used widely in air quality analysis and modeling, including
theapplication and evaluation of meteorological models, and as direct input
to air quality models. The vertical temperature structure (stability)
influences plume rise and expansion and thus the vertical exchange of
pollutants. Temperature also affects photolysis and chemical reaction rates.
Temperature is reported in degrees Celsius (°C)
or Kelvins (K).
Moisture
Like pressure, upper-air moisture
measurements suitable for air qualityapplications
are primarily obtained using radiosonde sounding systems. The sampling
frequency and vertical and temporal resolution of the moisture data are the
same as the other thermodynamic variables measured by these systems.
Moisture is most commonly measured directly as relative humidity (RH), and
is reported as percent RH or as dew-point temperature (Td) in °C (or frost
point temperature). Dew-point depression, the difference between temperature
and dew-point temperature (T - Td ), is also a commonly reported variable.
Some radiosonde sounding systems measure the wet-bulb temperature instead,
and determine RH and dew-point temperature through the psychrometric
relationship.
Upper-air
moisture profiles are used in the initialization and application of
meteorological models, and as direct input to air quality models. Moisture
data can be important to a successful meteorological modeling effort,
because the accurate simulation of convective development (clouds,
precipitation, etc.) depends on an accurate representation of the
three-dimensional moisture field. Upper-air moisture data are also useful to
the understanding of the formation and growth of aerosols, which grow
rapidly at high relative humidity (90 to 100 percent).
Mixing
Height For the purposes of this
guidance, mixing height is defined as the height of
the layer adjacent to the ground over which an emitted or entrained inert
non-buoyant tracer will be mixed (by turbulence) within a time scale of
about one hour or less (adapted from Beyrich [43] . This concept of a
mixing height was first developed for characterizing dispersion in a daytime
convective boundary layer (CBL). Since tracer measurements are impractical
for routine application, alternative methods are recommended for estimating
mixing heights based on more readily available data (Table 9-2). The
Holzworth method [44] is recommended for use when representative NWS
upper-air data are available. This procedure relies on the general
theoretical principle that the lapse rate is roughly dry adiabatic (no
change in potential temperature with height) in a well-mixed daytime
convective boundary layer (CBL); the Holzworth method is described in
Section 6.5.1. Other alternatives include using estimates of mixing heights
provided in CBL model output (references [45] and [46]).
Mixing heights derived from remote sensing measurements of turbulence or
turbulence related parameters are discussed in the following.
Turbulence,
or turbulence related measurements (e.g, backscatter measurements from a sodar
or refractive index measurements from a radar wind profiler) though not
surrogates for an inert tracer can sometimes be used to estimate mixing
heights since, under certain conditions, such measurements correlate with
the top of the mixed layer. In looking at these measurements, one attempts
to determine depth of the layer adjacent to the surface within which there
is continuous or intermittent turbulence; this is a non-trivial exercise
since turbulence varies considerably, not only with height, but with time
and location. This variability is dependent upon which processes
control/dominate the production of turbulence near the surface; these
processes are discussed in the following.
The
production of turbulent eddies during the daytime is dominated (under clear
sky conditions) by heating of the ground
surface and (under overcast conditions) by frictional drag. Daytime vertical
mixing processes can be vigorous (especially under convective -conditions)
and can produce a well mixed or nearly uniform vertical concentration
profile of an inert tracer. During the nighttime, there are several
processes that contribute to the production of turbulence including wind
shear (created near the ground by friction), variations in the geostrophic
wind, and the presence of a low-level jet (wind shear both below and above
the jet can enhance turbulence). Nighttime vertical mixing processes are
typically patchy and intermittent, and not capable of producing a well-mixed
uniform vertical concentration profile.
Table
9-2
Methods
for Determining Mixing Heights